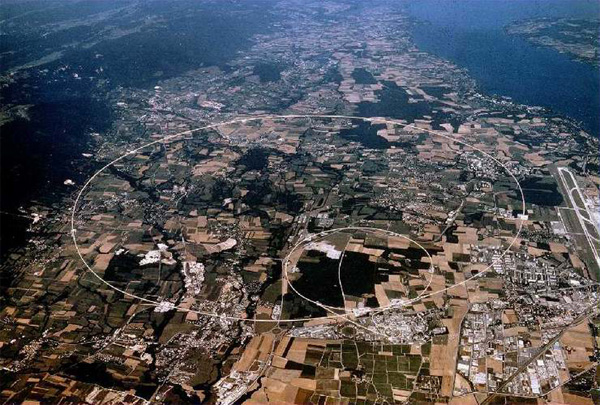
CERN The Large Hadron Collider is mostly
underground, as shown by the circle on this aerial view of the site on the
French-Swiss border.
The Future of Physics
Scientific American Magazine, February 2008
Edited by Andy Ross
The terascale is the realm of physics that comes into view when two
elementary particles smash together with a combined energy of around a
trillion electron volts, or one TeV. The machine that will take us to the
terascale — the ring-shaped Large Hadron Collider (LHC) at CERN — is now
nearing completion.
To ascend through the energy scales from electron
volts to the terascale is to travel from the domains of chemistry and
solid-state electronics (electron volts) to nuclear reactions (millions of
electron volts) to the territory that particle physicists have been
investigating for the past half a century (billions of electron volts).
What lies in wait for us at the terascale? No one knows.
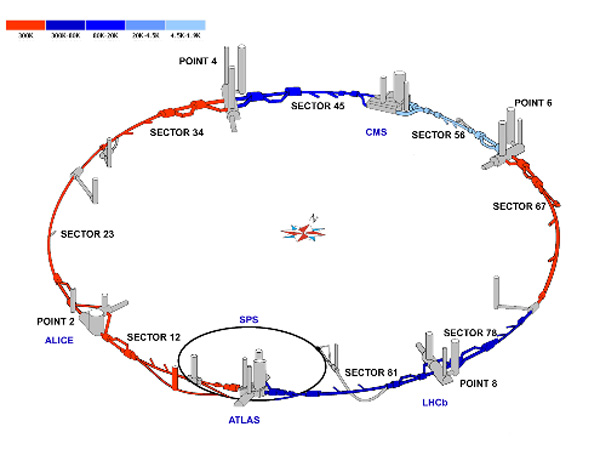
LHC cooldown status as of February 23, 2008
The Large Hadron Collider
By Graham P. Collins
Edited by Andy Ross
The Large Hadron Collider (LHC), a short drive from Geneva, will peer into
the physics of the shortest distances and the highest energies ever probed.
For a decade or more, particle physicists have been eagerly awaiting a
chance to explore the terascale domain. Significant new physics is expected
to occur at these energies, such as the elusive Higgs particle (believed to
be responsible for imbuing other particles with mass) and the particle that
constitutes the dark matter that makes up most of the material in the
universe.
The mammoth machine, after a nine-year construction period,
is scheduled to begin producing its beams of particles later this year. The
commissioning process is planned to proceed from one beam to two beams to
colliding beams, from lower energies to the terascale, from weaker test
intensities to stronger ones suitable for producing data at useful rates.
Each step will produce challenges for the more than 5,000 scientists,
engineers and students collaborating on the effort. The particle physics
community is eagerly awaiting the first results from the LHC.
To
break into the new territory that is the terascale, the LHC's basic
parameters outdo those of previous colliders in almost every respect. It
starts by producing proton beams of far higher energies than ever before.
Its nearly 7,000 magnets, chilled by liquid helium to less than two kelvins
to make them superconducting, will steer and focus two beams of protons
traveling within a millionth of a percent of the speed of light. Each proton
will have about 7 TeV of energy — 7,000 times a proton rest mass. When it is
fully loaded and at maximum energy, all the circulating particles will carry
energy roughly equal to the kinetic energy of about 900 cars traveling at
100 kilometers per hour.
The protons will travel in nearly 3,000
bunches, spaced all around the 27-kilometer circumference of the collider.
Each bunch of up to 100 billion protons will be the size of a needle, just a
few centimeters long and squeezed down to 16 microns in diameter (about the
same as the thinnest of human hairs) at the collision points. At four
locations around the ring, these needles will pass through one another,
producing more than 600 million particle collisions every second. The
collisions, or events, will occur between the quarks and gluons making up
the protons. The most cataclysmic of the smashups will release about 2 TeV.
Four giant detectors — the largest would roughly half-fill the Notre
Dame cathedral in Paris, and the heaviest contains more iron than the Eiffel
Tower — will track and measure the thousands of particles spewed out by each
collision occurring at their centers. Despite the detectors' vast size, some
elements of them must be positioned with a precision of 50 microns.
The nearly 100 million channels of data streaming from each of the two
largest detectors would fill 100,000 CDs every second. So instead of
attempting to record it all, the experiments will have what are called
trigger and data-acquisition systems, which act like vast spam filters,
immediately discarding almost all the information and sending the data from
only the most promising-looking 100 events each second to the LHC's central
computing system at CERN for archiving and later analysis.
A farm of
a few thousand computers at CERN will turn the filtered raw data into more
compact data sets organized for physicists to comb through. Their analyses
will take place on a grid network comprising tens of thousands of PCs at
institutes around the world, all connected to a hub of a dozen major centers
on three continents that are in turn linked to CERN by dedicated optical
cables.
In the coming months, all eyes will be on the accelerator.
The final connections between adjacent magnets in the ring were made in
November 2007, and the eight sectors are now being cooled almost to the
cryogenic temperature required for operation. After the operation of the
sectors has been tested, first individually and then together as an
integrated system, a beam of protons will be injected into one of the two
beam pipes that carry them around the machine's 27 kilometers.
The
series of smaller accelerators that supply the beam to the main LHC ring has
already been checked out. The first injection of the beam will be a critical
step, and the LHC scientists will start with a low-intensity beam to reduce
the risk of damaging LHC hardware. Only when they have carefully assessed
how that pilot beam responds inside the LHC and have made fine corrections
to the steering magnetic fields will they proceed to higher intensities. For
the first running at the design energy of 7 TeV, only a single bunch of
protons will circulate in each direction.
As the full commissioning
of the accelerator proceeds in this measured step-by-step fashion, problems
are sure to arise. The big unknown is how long the engineers and scientists
will take to overcome each challenge. If a sector has to be brought back to
room temperature for repairs, it will add months. The four experiments —
ATLAS, ALICE, CMS and LHCb — also have a lengthy process of completion ahead
of them, and they must be closed up before the beam commissioning begins.
When everything is working together at the design luminosity, as many as
20 events will occur with each crossing of the needlelike bunches of
protons. As little as 25 nanoseconds pass between one crossing and the next.
Product particles sprayed out from the collisions of one crossing will still
be moving through the outer layers of a detector when the next crossing is
already taking place. Individual elements in each of the detector layers
respond as a particle of the right kind passes through it. The millions of
channels of data streaming away from the detector produce about a megabyte
of data from each event, a petabyte every two seconds.
The trigger
system that will reduce this flood of data to manageable proportions has
multiple levels. The first level will receive and analyze data from only a
subset of all the detector's components, from which it can pick out
promising events. This level-one triggering will be conducted by hundreds of
dedicated computer boards. They will select 100,000 bunches of data per
second for more careful analysis by the next stage, the higher-level
trigger.
The higher-level trigger will receive data from all of the
detector's millions of channels. Its software will run on a farm of
computers, and with an average of 10 microseconds elapsing between each
bunch approved by the level-one trigger, it will have enough time to
reconstruct each event. It will project tracks back to common points of
origin and thereby form a coherent set of data for the particles produced by
each event.
The higher-level trigger passes about 100 events per
second to the hub of the LHC's global network of computing resources — the
LHC Computing Grid. A grid system combines the processing power of a network
of computing centers and makes it available to users who may log in to the
grid from their home institutes.
The LHC grid is organized into
tiers. Tier 0 is at CERN itself and consists in large part of thousands of
commercially bought servers, both PC-style boxes and, more recently, blade
systems looking like black pizza boxes, stacked in row after row of shelves.
Computers are still being purchased and added to the system. The data passed
to Tier 0 by the LHC data-acquisition systems will be archived on magnetic
tape.
Tier 0 will distribute the data to the 12 Tier 1 centers, which
are located at CERN itself and at 11 other major institutes around the
world. Thus, the unprocessed data will exist in two copies, one at CERN and
one divided up around the world. Each of the Tier 1 centers will also host a
complete set of the data in a compact form structured for physicists to
carry out many of their analyses.
The full LHC Computing Grid also
has Tier 2 centers, which are smaller computing centers at universities and
research institutes. Computers at these centers will supply distributed
processing power to the entire grid for the data analyses.
The LHC
has experienced some setbacks along the way. Last March a magnet of the kind
used to focus the proton beams just ahead of a collision point (called a
quadrupole magnet) suffered a serious failure during a test of its ability
to stand up to the forces that could occur if its coils lost their
superconductivity (a mishap called quenching). Part of the supports of the
magnet had collapsed under the pressure of the test, producing a loud bang
like an explosion.
These magnets come in groups of three, to squeeze
the beam first from side to side, then in the vertical direction, and
finally again side to side, a sequence that brings the beam to a sharp
focus. The LHC uses 24 of them, one triplet on each side of the four
interaction points. At first the LHC scientists did not know if all 24 would
need to be removed from the machine and brought above-ground for
modification, a time-consuming procedure that could have added weeks to the
schedule. The problem was a design flaw. CERN and Fermilab researchers
worked feverishly, identifying the problem and coming up with a strategy to
fix the undamaged magnets in the accelerator tunnel.
In June, CERN
director general Robert Aymar announced that he had to postpone the
scheduled start-up of the accelerator from November 2007 to spring 2008. The
beam energy is to be ramped up faster to try to stay on schedule for doing
physics by July.
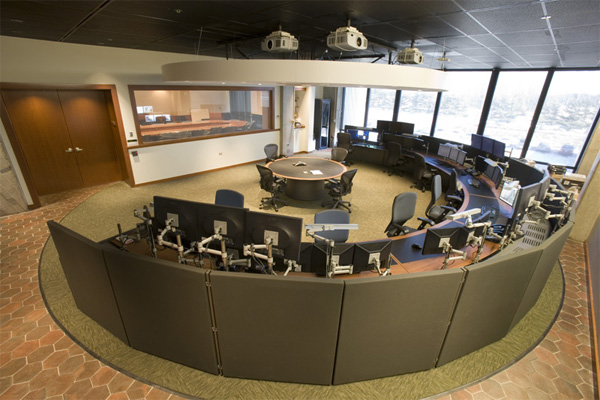 The LHC
Remote Operations Center
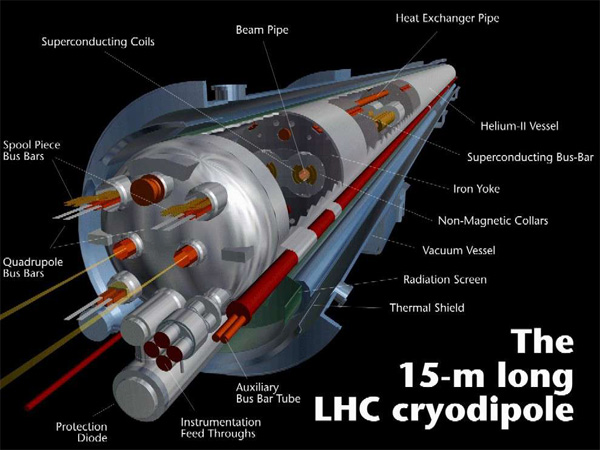 One of the
LHC cryogenic superconducting dipole magnets
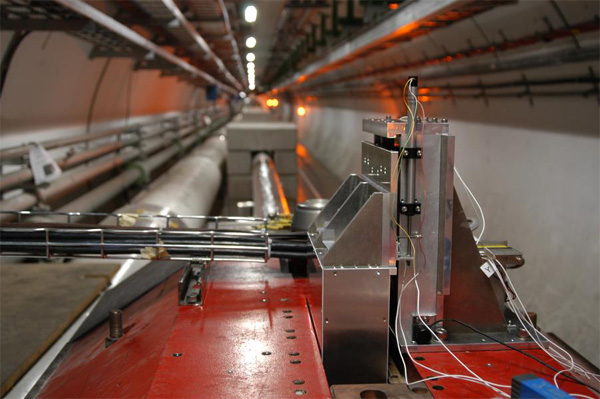 A view along
the LHC main tunnel
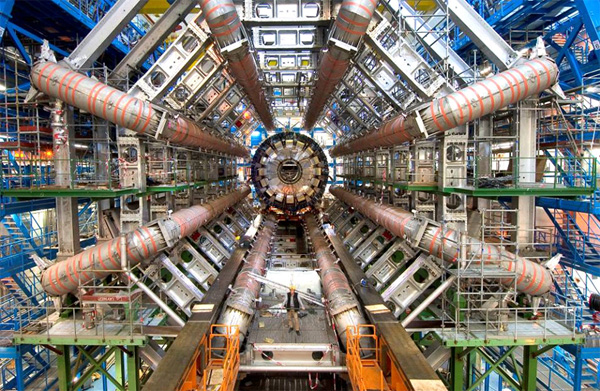 The LHC
ATLAS detector under construction, with an engineer in the foreground
The Coming Revolutions in Physics
By Chris Quigg
Edited by Andy Ross
When physicists are asked to give a short answer to the question of why we
are building the Large Hadron Collider (LHC), we usually reply "Higgs" — the
Higgs particle, the last remaining undiscovered piece of our current theory
of matter. But the full story is more interesting. The new collider provides
the greatest leap in capability of any instrument in the history of particle
physics.
In this new world, we expect to learn what distinguishes two
of the forces of nature — electromagnetism and the weak interactions — with
broad implications for our conception of the everyday world. We will gain a
new understanding of simple and profound questions: Why are there atoms? Why
chemistry? What makes stable structures possible?
The search for the
Higgs particle is only the first step. Beyond it lie phenomena that may
clarify why gravity is so much weaker than the other forces of nature and
that could reveal what the unknown dark matter that fills the universe is.
Even deeper lies the prospect of insights into the different forms of
matter, the unity of outwardly distinct particle categories and the nature
of spacetime. The LHC will help us refine these questions.
The
Standard Model of particle physics can explain much about the known world.
The main elements of the Standard Model fell into place during the 1970s and
1980s. Yet even as the Standard Model has gained ever more experimental
support, a growing list of phenomena lies outside its purview, and new
theoretical ideas have expanded our conception of what a richer and more
comprehensive worldview might look like. Taken together, the continuing
progress in experiment and theory point to a very lively decade ahead.
Our current conception of matter comprises two main particle categories,
quarks and leptons, together with three of the four known fundamental
forces, electromagnetism and the strong and weak interactions. Gravity is,
for the moment, left to the side. Quarks, which make up protons and
neutrons, generate and feel all three forces. Leptons, the best known of
which is the electron, are immune to the strong force. What distinguishes
these two categories is a property akin to electric charge, called color.
Quarks have color, and leptons do not.
The guiding principle of the
Standard Model is that its equations are symmetrical. The equations remain
unchanged when you change the perspective from which they are defined, even
when the perspective shifts by different amounts at different points in
space and time. The symmetry of the equations places very tight constraints
on them. These symmetries beget forces that are carried by special particles
called bosons.
In the Standard Model, the symmetry of the equations
dictates the interactions among particles that the theory describes. For
instance, the strong nuclear force follows from the requirement that the
equations describing quarks must be the same no matter how one chooses to
define quark colors. The strong force is carried by eight particles known as
gluons. The other two forces, electromagnetism and the weak nuclear force,
are the electroweak forces and are based on a different symmetry. The
electroweak forces are carried by a quartet of particles: the photon, Z
boson, W+ boson and W– boson.
The theory of the electroweak forces
was formulated by Sheldon Glashow, Steven Weinberg and Abdus Salam. The weak
force, which is involved in radioactive beta decay, does not act on all the
quarks and leptons. Each of these particles comes in left-handed and
right-handed varieties, and the beta-decay force acts only on the
left-handed ones — a striking fact still unexplained 50 years after its
discovery.
In the initial stages of its construction, the theory had
two essential shortcomings. First, it foresaw four long-range force
particles—referred to as gauge bosons—whereas nature has but one: the
photon. The other three have a short range, less than about 10–17 meter.
According to Heisenberg’s uncertainty principle, this limited range implies
that the force particles must have a mass approaching 100 GeV. The second
shortcoming is that the family symmetry does not permit masses for the
quarks and leptons, yet these particles do have mass.
The way out
here is to recognize that a symmetry of the laws of nature can be broken.
The needed theoretical apparatus was worked out in the 1960s by Peter Higgs,
Robert Brout, François Englert and others. The inspiration came from
superconductivity, in which certain materials carry electric current with
zero resistance at low temperatures. Although the laws of electromagnetism
themselves are symmetrical, the behavior of electromagnetism within the
superconducting material is not. A photon gains mass within a
superconductor, thereby limiting the intrusion of magnetic fields into the
material.
This phenomenon is a prototype for the electroweak theory.
If space is filled with a type of superconductor that affects the weak
interaction rather than electromagnetism, it gives mass to the W and Z
bosons and limits the range of the weak interactions. This superconductor
consists of particles called Higgs bosons. The quarks and leptons also
acquire their mass through their interactions with the Higgs boson. By
obtaining mass in this way, these particles remain consistent with the
symmetry requirements of the weak force.
The paradigm of quark and
lepton constituents interacting by means of gauge bosons completely revised
our conception of matter and pointed to the possibility that the strong,
weak and electromagnetic interactions meld into one when the particles are
given very high energies. The electroweak theory shows how the quarks and
leptons might acquire masses but does not predict what those masses should
be. The electroweak theory is similarly indefinite in regard to the mass of
the Higgs boson itself. Many of the outstanding problems of particle physics
and cosmology are linked to the question of exactly how the electroweak
symmetry is broken.
Encouraged by a string of promising observations,
theorists began to take the Standard Model seriously enough to begin to
probe its limits. Toward the end of 1976 Benjamin W. Lee, Harry B. Thacker,
and I devised a thought experiment to investigate how the electroweak forces
would behave at very high energies. We imagined collisions among pairs of W,
Z and Higgs bosons. At the time of our work, not one of these particles had
been observed.
We noticed a subtle interplay among the forces
generated by these particles. Extended to very high energies, our
calculations made sense only if the mass of the Higgs boson were not too
large — the equivalent of less than 1 TeV. If the Higgs is lighter than 1
TeV, weak interactions remain feeble and the theory works reliably at all
energies. If the Higgs is heavier than 1 TeV, the weak interactions
strengthen near that energy scale and all manner of exotic particle
processes ensue. This mass threshold means that something new is to be found
when the LHC turns the thought experiment into a real one.
Experiments may already have observed the influence of the Higgs. The
uncertainty principle implies that particles such as the Higgs can exist for
moments too fleeting to be observed directly but long enough to leave a
subtle mark on particle processes. The Large Electron Positron collider at
CERN, the previous inhabitant of the tunnel now used by the LHC, detected
the work of such an unseen hand. Comparison of precise measurements with
theory strongly hints that the Higgs exists and has a mass less than about
192 GeV.
For the Higgs to weigh less than 1 TeV, as required, poses
an interesting riddle. Quantities such as mass are modified by quantum
effects. Just as the Higgs can exert a behind-the-scenes influence on other
particles, other particles can do the same to the Higgs. Those particles
come in a range of energies, and their net effect depends on where precisely
the Standard Model gives way to a deeper theory. If the model holds all the
way to 1015 GeV, where the strong and electroweak interactions appear to
unify, particles with truly titanic energies act on the Higgs and give it a
comparably high mass. So why does it appear to have a mass of no more than 1
TeV?
This tension is known as the hierarchy problem. One resolution
would be a precarious balance of additions and subtractions of the
contending contributions of different particles. Physicists are suspicious
of immensely precise cancellations that are not mandated by deeper
principles. It seems likelier that both the Higgs boson and other new
phenomena will be found with the LHC.
Theorists have explored many
ways to resolve the hierarchy problem. Supersymmetry supposes that every
particle has an as yet unseen superpartner that differs in spin. If nature
were exactly supersymmetric, the masses of particles and superpartners would
be identical, and their influences on the Higgs would cancel each other out
exactly. But in that case, physicists would have seen the superpartners by
now. So if supersymmetry exists, it must be a broken symmetry. The net
influence on the Higgs could still be acceptably small if superpartner
masses were less than about 1 TeV, within reach of the LHC.
Another
option, called technicolor, supposes that the Higgs boson is not truly a
fundamental particle but is built out of as yet unobserved constituents. If
so, the Higgs is not fundamental. Collisions at energies around 1 TeV would
allow us to look within it and thus reveal its composite nature. Like
supersymmetry, technicolor implies that the LHC will set free a veritable
menagerie of exotic particles.
One more piece of evidence points to
new phenomena on the TeV scale. The dark matter that makes up the bulk of
the material content of the universe appears to be a novel type of particle.
If this particle interacts with the strength of the weak force, then the big
bang would have produced it in the requisite numbers as long as its mass
lies between approximately 100 GeV and 1 TeV.
Opening the TeV scale
to exploration means entering a new world of experimental physics. Making a
thorough exploration of this world is the top priority for accelerator
experiments. The answers will not only be satisfying for particle physics,
they will deepen our understanding of the everyday world.
The Guardian CERN page
Guardian
online, June/July 2008
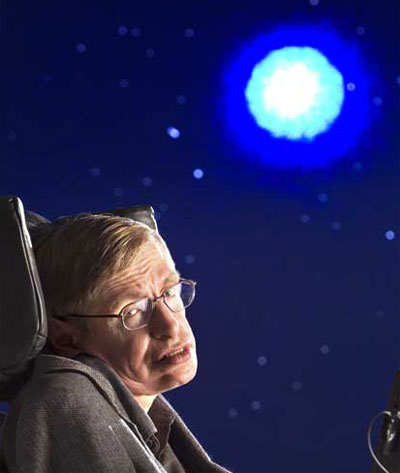
Guardian Stephen Hawking
"The Large Hadron Collider at CERN will smash particles together to
recreate the moments after the big bang. Some theories of spacetime suggest
the particle collisions might create mini black holes. If that happened, I
have proposed that these black holes would radiate particles and disappear.
If we saw this at the LHC, it would open up a new area of physics, and I
might even win a Nobel prize. But I'm not holding my breath." —
Stephen Hawking
"Cathedrals were designed to celebrate the glory
of God as manifested through the human spirit in words, music and art. The
LHC has been engineered to celebrate and proclaim the glory of the natural
world, and of our remarkable ability to comprehend it, as manifested through
experimental science." —
Lawrence Krauss
Plus articles by:
Brian Cox
A.C. Grayling
Michio Kaku
Martin Rees and others
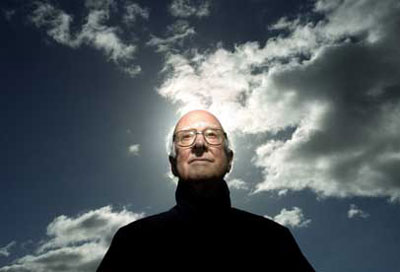
Guardian Peter Higgs
Father of the 'God particle' The Higgs boson is the particle that is
thought to give everything else in the universe mass. Its theistic nickname
was coined by Leon Lederman, but Higgs himself is no fan of the label. "I
find it embarrassing because, though I'm not a believer myself, I think it
is the kind of misuse of terminology which I think might offend some
people."
Genesis machine poised to end quest for 'God particle' "I sincerely
hope I'm not the only one who's at least slightly worried about this mad
scientist Peter Higgs and his 'Genesis machine'" —
a reader
A Bluffer's Guide
A graphic guide to the LHC, how it will work and the physics that lies
behind it all
Da LHC
is Superduper Fly — rap video
Smashing Idea
By William Booth Washington Post, September 11, 2008
Edited by Andy Ross
It is the biggest machine ever built. Everyone says it looks like a movie
set for a corny James Bond villain. They are correct. The machine is
attended by brainiacs wearing hard hats and running around on catwalks. They
are looking for the answer to the question: Where does everything in the
universe come from? Price tag: $8 billion plus.
The world's largest
particle accelerator is buried deep in the earth beneath herds of placid
dairy cows grazing on the Swiss-French border. The thing has been under
construction for years, like the pyramids. Its centerpiece is a circular
17-mile tunnel that contains a pipe swaddled in supermagnets refrigerated to
crazy-low temperatures, colder than deep space.
The idea is to set
two beams of protons traveling in opposite directions around the tunnel,
redlining at the speed of light, generating wicked energy that will mimic
the cataclysmic conditions at the beginning of time, then smashing into each
other in a furious re-creation of the Big Bang — this time recorded by giant
digital cameras.
Wednesday, they fired this sucker up ...
AR This is so exciting. I can hardly wait for
the results — what will the Higgs look like?
The Higgs Boson
By Anna Kucirkova, May 2018
Edited by Andy Ross
|